Making it work
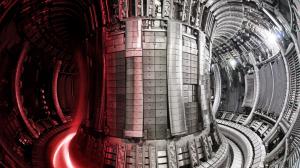
Although different isotopes of light elements can be paired to achieve fusion, the deuterium-tritium (D-T) reaction has been identified as the most achievable and the most efficient.
At the core of fusion science is plasma physics.
At extreme temperatures, electrons are separated from nuclei and a gas becomes a plasma—an ionized state of matter similar to a gas. Composed of charged particles (positive nuclei and negative electrons), plasmas are very tenuous environments, nearly one million times less dense than the air we breathe. Fusion plasmas provide the environment in which light elements can fuse and yield energy.
Three conditions must be fulfilled to achieve fusion in a laboratory: very high temperature (to provoke high-energy collisions); sufficient plasma particle density (to increase the likelihood that collisions do occur); and sufficient confinement time (to hold the plasma, which has a propensity to expand, within a defined volume).
In ITER, fusion will be achieved in a tokamak device that uses magnetic fields to contain and control the hot plasma. The plasma particles are heated—that is, sped up—by different types of auxiliary heating methods. The fusion between deuterium and tritium (D-T) nuclei produces one helium nucleus, one neutron, and great amounts of energy.
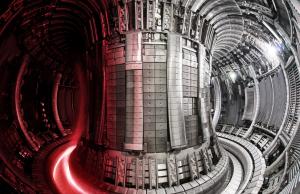
Three, two, one ... We have plasma! Inside the European JET Tokamak, both during and after operation. Photo: UKAEA
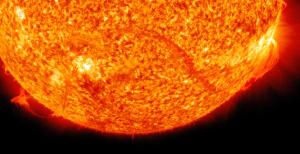
In terms of temperature and physical phenomena, the outer region of the plasma is "very similar to the Sun's corona," says Robin Barnsley. © Soho-Nasa/Esa
Capturing the energy
The helium nucleus carries an electric charge that is subject to the magnetic fields of the tokamak, keeping it confined within the plasma where it contributes to its continued heating. However, approximately 80 percent of the energy produced is carried away from the plasma by the neutron which has no electrical charge and is therefore unaffected by magnetic fields. The neutrons will be absorbed by the surrounding walls of the tokamak, where their kinetic energy will be transferred to the walls as heat.
In ITER, this heat will be captured by cooling water circulating in the vessel walls and eventually dispersed through cooling towers. In the type of fusion power plant envisaged for the second half of this century, the heat will be used to produce steam and—by way of turbines and alternators—electricity.
In terms of sheer scale, the energy potential of the fusion reaction is superior to all other energy sources that we know on Earth. Fusing atoms together in a controlled way releases nearly four million times more energy than a chemical reaction such as the burning of coal, oil or gas and four times more than nuclear fission.
Remaining challenges
ITER is designed to achieve and sustain fusion reactions at a scale that will enable the study of a controlled "burning" (self-heating) plasma. However, achieving sustained fusion reactions is only one piece of the puzzle. To harness fusion as a practical energy source, several key challenges must be addressed. ITER will contribute to addressing each of them, in an integrated manner, but further R&D is a prerequisite to delivering a credible design for a next-phase demonstration reactor.
Materials resistant to extreme conditions: The intense flux of high-energy neutrons and other particles generated during fusion reactions subject the reactor's structural materials to extreme conditions. Finding materials capable of withstanding these conditions while maintaining structural integrity is a top priority. These materials must be compatible both with the requirements of plasma purity, and also with the harsh thermal, radiation and vacuum environments inside the reactor. ITER employs advanced materials in its design, but ITER's efforts must be supplemented with materials science R&D to identify materials that can withstand the rigors of long-term fusion operation.
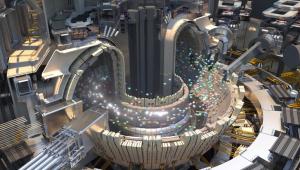
Visualization courtesy of Jamison Daniel, Oak Ridge Leadership Computing Facility
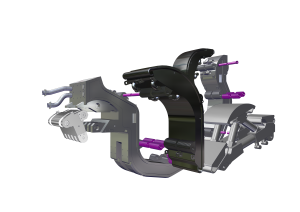
Heat exhaust management in the divertor region: One of the primary challenges in fusion reactor design is managing the heat and particle exhaust from the plasma. In tokamaks like ITER, this is accomplished through a component called the divertor, which extracts heat and particles from the plasma and protects the reactor's walls from damage. The extreme power density conditions in the divertor region present significant engineering challenges. ITER is pioneering novel divertor designs (image) and cooling techniques at the present limits of technology. Ongoing research is needed to optimize heat exhaust management in future reactors.
Remote handling for maintenance: Fusion reactors operate in harsh environments that are inaccessible to human operators. As a result, maintenance and repair tasks must be performed remotely using advanced robotic systems. ITER is developing state-of-the-art remote handling technologies to address the challenge, but ensuring the reliability and efficiency of these systems in a practical fusion reactor environment remains a significant engineering hurdle. Research efforts are focused on enhancing the dexterity, agility and autonomy of remote handling systems to enable efficient maintenance operations in future fusion power plants and to optimize the size of associated maintenance facilities.
Tritium fuel cycle: Tritium, a radioactive isotope of hydrogen, is a key fuel for deuterium-tritium (D-T) fusion reactors. However, tritium is not naturally abundant and must be produced artificially. Additionally, tritium has a relatively short (12.3 year) half-life. Developing efficient tritium breeding and extraction techniques is essential to sustaining fusion reactions in a practical D-T reactor. ITER is conducting experiments to test tritium breeding blankets and fuel cycle technologies, but further research is needed to demonstrate the feasibility of large-scale tritium production and recycling.
Efficient heat removal for electricity generation: While fusion produces intense energy, the physical structure of tokamak fusion reactors is that of a geometric heat source, poorly configured to efficiently remove the heat produced from the fusion reaction. Traditional heat removal designs used in fossil fuel or nuclear fission plants interface directly with the heat generation process, whereas in a fusion reactor any such interface would disrupt the plasma and stop the fusion reaction. ITER's design will demonstrate heat removal, but more R&D is needed to raise the efficiency for practical electricity generation.
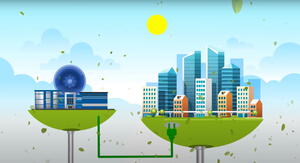
A fusion reaction is about four million times more energetic than a chemical reaction such as the burning of coal, oil or gas.